Extending the Building Blocks of Materials Science: What Viruses have to Offer.
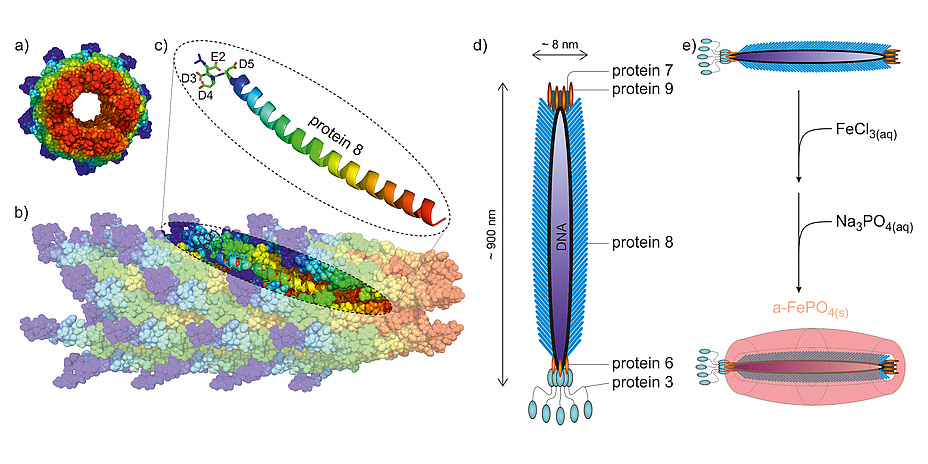
Whereas we are still struggling with large-scale production of three-dimensional, nano-sized materials, nature perfected this task in viruses long before the dawn of humanity. Bionanotechnologists use these viral structures as templates for the synthesis of nanostructured inorganic materials with new outstanding properties.
One of the major challenges of the 21st century is the need for sustainable energy conversion and energy storage devices. Therefore, we have to identify novel materials for energy storage and at the same time, we have to develop low-energy consuming and sustainable strategies to prepare such materials on a large scale. The use of biological templates represents an elegant way to alter the morphology and properties of materials synthesized by e.g. aqueous precipitation routes. Using this approach, we prepared highly an-isotropic cathode materials for Li-ion batteries with surface-dominated properties and particle shapes resembling the template. Such cathode materials are often amorphous, a state not easily attainable by other synthesis routes. The material properties of the amorphous state are very different from those of their crystalline counterparts and are, if the particles are nano-sized, highly dependent on the shape, i.e. morphology, and concomitantly, on the surface-effects. Thus, the material properties can be changed and ultimately fine-tuned using this fairly novel and unconventional method.
Biotemplation
There is plenty of space on the surface of a virus. We use the bacteriophage M13, a virus which exclusively infects Escherichia coli, as a biological template for the synthesis of iron phosphate nanowires. Iron phosphate (FePO4) is a very promising active material used in battery cathodes. This material offers high-charge storage capacities and is very ecofriendly, consisting of highly abundant elements. However, it has certain drawbacks such as low electronic and Li-ionic conductivities – two quantities that essentially define the performance of cathode materials. Currently, this material is used in batteries but requires an energy-demanding synthesis route which yields micro- to nano-sized carbon coated LiFePO4, which is the discharge product of FePO4. Increasing the surface-to-volume ratio significantly reduces diffusion lengths [1], [2] and increases performance, whereas carbon-coating enables efficient electronic transport thereby enabling fast redox reactions to occur. Nevertheless, for crystalline (Li)FePO4, a classic example of a two-phase material [3], the crystallinity grants an almost constant potential during charge/discharge but also restricts Li-ion diffusion to one-dimensional (1D) channels that are easily blocked by defects. For amorphous (Li)FePO4 the situation might be very different, and the Li-ion conductivity is anticipated to be much higher as the ionic transport is no longer restricted to 1D-channels.
The filamentous bacteriophage M13 can easily be genetically engineered to harbour specific properties. Most importantly, thousands of copies of protein 8 foremost assemble its proteinaceous coat (capsid). Thus, by genetically modifying the genetic code for protein 8, we can easily control the surface properties of the bacteriophage as these properties depend on the specific surface-exposed amino acids. To use this bacteriophage for our synthesis, we mutated the genetic information coding for protein 8 so that it would carry four acidic amino acids at its N-terminal tail (Figure 1a blue regions). These surface-protruding regions show a pattern of four consecutive acidic amino acids, namely glutamic acid (E) followed by three residues of aspartic acid (D), which enables the surface of the bacteriophage to electrostatically bind cations.
Green Synthesis of Cathode Materials
Here we used the modified bacteriophage M13 as a template for the synthesis of the charged cathode material FePO4. In a simple aqueous synthesis process the bacteriophage is first incubated with an Fe3+ containing solution to allow its surface to coordinate sufficient iron cations (Figure 2a).
In a subsequent step, the addition of a PO43- containing solution leads to the rapid precipitation of amorphous FePO4 due to its very low solubility product. Most importantly, the precipitates adopt the filamentous form of the template resulting in entangled nanowires of amorphous FePO4 (Figure 2b). In this manner, amorphous FePO4 nanowires can be synthesized by employing a biotemplated aqueous precipitation route at room temperature. Without the restrictions imposed by the crystal structure of LiFePO4, the Li-ion conductivity is much higher. Purification by filtration and subsequent drying at comparatively low temperatures renders this material ready to use for Li-ion batteries.
Figure 2: Transmission electron microscopy images of the modified bacteriophage M13 after incubation in iron chloride solution (a) and after subsequent precipitation as amorphous FePO4 nanowires (b).
Performance
Li-ion cells with cathodes of a-LiFePO4 offer a great advantage compared to cells using its crystalline counterpart. As the potential of this cathode highly depends on the state of charge, which is the amount of Li stored in the cathode material, for this type of cells the state of charge can be determined easily. In c-LiFePO4 with its very flat plateau, estimation of the SOC is difficult, especially if the cell is not at rest, i.e., if the cell is delivering power.
As intimated above, the diffusion of Li-ions is highly influenced by the crystal structure of LiFePO4, which allows the ions to diffuse almost exclusively within the one-dimensional channels (Figure 3).
Figure 3: Figure 3: Crystal structure of lithium iron phosphate, LiFePO4. The octahedrally coordinated Fe-ions (black) form a sheet connected by tetrahedrally coordinated P (purple). Lithium ions (green) preferentially move within the formed 1-dimensional channels by jump processes (yellow arrows).
If the long-range order of the atoms within the material is abolished, so are the restrictions imposed by the arrangements, and the Li-ions are facing a surrounding with a less restrictive degree of freedom i.e. 3D diffusion is rendered possible. Many cathode materials suffer from structural disorder; however, this is not the case for LiFePO4. It is well known that disorder and vacancies can increase conductivity by several orders of magniture and amorphous LiFePO4 is one of the very few cathode materials for which this holds. Without the restrictions imposed by the crystal structure of LiFePO4, the Li-ion conductivity is much higher. Tests of chemically lithiated a-FePO4 (a-LiFePO4) by impedance spectroscopy clearly show the outstanding increase of the conductivity, σDC, compared to its crystalline counterparts; even so if the crystallites are nanosized (Figure 4).
Figure 4: Temperature dependent conductivity, σDC∙T, of crystalline LiFePO4 and biotemplated amorphous LiFePO4 as a function of the inverse temperature in an Arrhenius representation. Clearly, the activation barrier for conduction is significantly decreased in a-LFP (0.36 eV) compared to c-LFP (0.6-0.7 eV)6,7 whereas the conductivity at room temperature is increased by three orders of magnitude.
The ionic conductivity shows a typical increase with temperature and can be well described by the famous Arrhenius relation. Close to room temperature, the conductivity of the biotemplated amorphous LiFePO4 is increased by almost three orders of magnitude even compared to crystalline LiFePO4 with particle sizes of 50 nm. Interestingly, the activation energies associated with the mean energy barrier ions have to overcome in order to diffuse are significantly reduced from ~0.6 eV to ~0.36 eV, which corroborates well with theory.
Outlook
The use of viruses as building blocks in materials science extends the possibilities of fine-tuning material properties by shaping nanosized materials. With the synthesis of amorphous FePO4 nanowires we are just scratching at the surface of what is possible with these templates. With this tool at hand, the synthesis of nanocomposite materials is just one step away from the synthesis of isotropic nanomaterials. The tips of the bacteriophage M13 composed by either protein 7 & 9 or 6 & 3 can also be altered in a way to have specific binding capabilities for various materials. Using these additional modification attributes, the formation of 3D organized nano scaffolds will be possible.
Financial support from the Austrian Federal Ministry of Science, Research and Economy and the National Foundation for Research, Technology and Development (CDLaboratory of Lithium Batteries: Ageing Effects, Technology and New Materials) is gratefully acknowledged.
- Aricò, A. S.; Bruce, P.; Scrosati, B.; Tarascon, J.-M.; van Schalkwijk, W., Nanostructured Materials for Advanced Energy Conversion and Storage Devices. Nature Materials 2005, 4, 366-377.
- Wilkening, M.; Lyness, C.; Armstrong, A. R.; Bruce, P. G., Diffusion in Confined Dimensions: Li+ Transport in Mixed Conducting TiO2−B Nanowires. The Journal of Physical Chemistry C 2009, 113, 4741-4744.
- A. K. Padhi, K. S. N. a. J. B. G., Phospho-Olivines as Positive-Electrode Materials for Rechargeable Lithium Batteries. Journal of The Electrochemical Society 1997, 144, 1188.
- Morag, O.; Sgourakis, N. G.; Baker, D.; Goldbourt, A., The NMR–Rosetta Capsid Model of M13 Bacteriophage Reveals a Quadrupled Hydrophobic Packing Epitope. Proceedings of the National Academy of Sciences 2015, 112, 971-976.
- Schrodinger, LLC, The PyMol Molecular Graphics System, Version 1.8. 2015.
- Shahid, R.; Murugavel, S., Particle Size Dependent Confinement and Lattice Strain Effects in LiFePO4. Physical Chemistry Chemical Physics 2013, 15, 18809-18814.
- Zaghib, K.; Mauger, A.; Goodenough, J. B.; Gendron, F.; Julien, C. M., Electronic, Optical, and Magnetic Properties of LiFePO4: Small Magnetic Polaron Effects. Chemistry of Materials 2007, 19, 3740-3747.
Kontakt
Bernhard GADERMAIER
BSc MSc
Institute for Chemistry and Technology of Materials
Stremayrgasse 9
8010 Graz
Phone: +43 316 873 32326
bernhard.gadermaier @tugraz.at